Across the past century science has reached an understanding of the cosmos that is as profound as it is precise.
Einstein’s general theory of relativity provides a framework that describes the structure of spacetime at the largest observable scales—as well as the existence and behavior of compact objects such as black holes and novel phenomena such as gravitational waves. Quantum mechanics and particle physics help explain the formation of stars, how they shine, their deaths as supernovae and the way they enrich the universe with chemical elements across cosmic time. Physicists and astronomers have determined the universe’s expansion rate, the relative abundances of its bulk constituents and a host of other key cosmic parameters to a precision of better than a few percent. And they have used this knowledge to account for some 13.8 billion years of history, gauging the cosmic conditions that prevailed all the way back to the first billionth of a second after the big bang.
Much remains unknown, such as the true nature of the dark matter that dominates gravity inside galaxies and the dark energy that drives the universe’s accelerating expansion. Yet even for these great mysteries, we can observe and assess their large-scale effects with extraordinary clarity.
On supporting science journalism
If you’re enjoying this article, consider supporting our award-winning journalism by subscribing. By purchasing a subscription you are helping to ensure the future of impactful stories about the discoveries and ideas shaping our world today.
This modern era of high precision stems in part from studies of the oldest light accessible to us—the cosmic microwave background (CMB). The CMB is the big bang’s afterglow from circa 380,000 years after the universe’s fiery birth. Back then space was filled with a hot, dense, opaque fog of ionized particles. As the universe expanded and cooled, however, electrons and protons combined to make hydrogen atoms, clearing the fog in a cosmic instant and freeing light to burst forth. Attenuated across vast gulfs of time and space, that light appears to us today as faint wisps of microwaves emanating from the entire sky, forming a two-dimensional snapshot of the universe as it was in that ancient epoch. Observed patterns in the CMB—as well as in the large-scale distribution of galaxies—are diagnostic, to a remarkable degree, of a plethora of cosmic properties and form a cornerstone of our most cherished theories.
But after the CMB’s flash of boundless light, a great darkness fell because no stars yet existed to shine. These were the cosmic dark ages, and our most powerful telescopes have yet to pierce their depths. The cosmic dark ages were eventually ended by the cosmic dawn, when much of the hydrogen suffusing all of space gradually coalesced under gravity to kindle the first stars and assemble the first galaxies. Theoretical models reinforced by computer simulations suggest stars would not arrive for somewhere between a few tens of millions to 100 million years. Before that the only plausible luminous objects would have been a hypothetical population of primordial black holes, which, while lightless themselves, would be wreathed by incandescent vortices of infalling matter.
The exact nature and timing of these events is undetermined, but we do know with certainty that the emergence of the first stars and galaxies sparked another epochal transition across the next billion years or so of cosmic history.
Although light had now returned to the cosmos, immense volumes of lingering primordial hydrogen still stifled much of its spread. But as those first luminous objects shined, their intense emissions of ultraviolet and x-ray radiation heated up the gas and broke the hydrogen atoms back into constituent electrons and protons. This epoch of reionization returned all that ordinary, electrically neutral matter to the ionized, plasmatic state it had occupied long before, less than a half-million years after the big bang, when it was hot and dense. Yet after hundreds of millions of years of cosmic expansion, the universe had now grown so vast that this ionized material was diluted. Stretched thin through space, it remained essentially transparent to most forms of light, constituting the intergalactic medium and ensuring that starlight would stream freely through the cosmos forevermore.
Looking beyond Limits
The properties of the first stars, galaxies and black holes responsible for reionization are still unknown, because these objects are too far away and too dim for our present telescopes to see—but perhaps not for much longer. New observatories, such as the James Webb Space Telescope (JWST), can look almost unfathomably deep into space—and because light takes time to reach us, as with all far-seeing telescopes, JWST’s images show sources when they were younger—far younger. JWST, in fact, allows us to see galaxies all the way back to when the universe was a 330-million-year-old infant, merely a few percent of its current age of 13.8 billion years.

The youthful universe as revealed by observations and theoretical calculations is very different from the mature and evolved version we now inhabit. The cosmos was denser, yet star-forming regions were scattered more sparsely in space. Galaxies were shrunken compared with those of today because they formed hierarchically, starting from smaller, denser building blocks. The stars themselves were different, too, with their first generation being composed almost entirely of pristine hydrogen and helium. Heavier elements would only come later, fused into existence from those first stars’ thermonuclear fires. This lack of heavy elements, it is thought, allowed the first stars to be much more massive than those today—so massive that some among them would end their lives in cataclysmic explosions that were far larger than modern-day supernovae, spreading their heavy-element ashes to enrich future stellar generations.
Yet for all that JWST can see, much is still hidden. It has yet to definitively glimpse light from the first stars. And it can only detect the brightest of the earliest galaxies, while the far dimmer first star-forming regions and supernovae predicted to be the most typical early sources of light remain invisible, well below its detection thresholds. Even a forthcoming new generation of extremely large ground-based telescopes with light-gathering mirrors approaching 40 meters in diameter will not be able to gaze deeper back in space and time to the onset of star formation.
Remarkably, human ingenuity has found a new way to probe deeper into space and time than such gargantuan traditional telescopes can ever reach.
The technique does not rely on directly recording the light of the very first stars but rather uses exquisitely sensitive radio telescopes to seek the imprints they made upon the surrounding primordial hydrogen gas that filled intergalactic space at that time. Once again, the light from the CMB is crucial to unraveling these deep mysteries, but instead of offering a two-dimensional snapshot of the cosmos as it was 380,000 years after the big bang, the new technique uses complex contrasts between primordial hydrogen and the CMB to create a dynamic, three-dimensional map of the early universe’s growth across most of its first billion years of existence. This could allow astronomers to see all the way back to cosmic dawn to glimpse the emergence of the first stars, galaxies and black holes from the cosmic dark ages.
A 21-cm Cosmic Tapestry
A spectral transition of hydrogen atoms resonates with CMB photons at a wavelength of 21 centimeters as they pass through, distorting the CMB black body spectrum and providing a way to probe the primordial gas that filled intergalactic space during the cosmic dawn and the epoch of reionization.
This unconventional way of mapping the universe is based on an atomic transition of hydrogen associated with a change in the relative alignment between the spins of the proton and the electron that make the atom. This so-called spin-flip transition involves a photon of the radio frequency of 1.4 gigahertz, which corresponds to the wavelength of 21 cm, and it is thus also referred to as the “21-cm signal.” The spin-flip transition manifests in two ways: hydrogen atoms colder than the CMB absorb a 21-cm photon and partially block the CMB’s propagation, whereas hydrogen atoms hotter than the CMB emit a 21-cm photon and add their brightness to the background. Radio telescopes can detect and map these signals from neutral hydrogen as distortions upon the CMB black body spectrum.
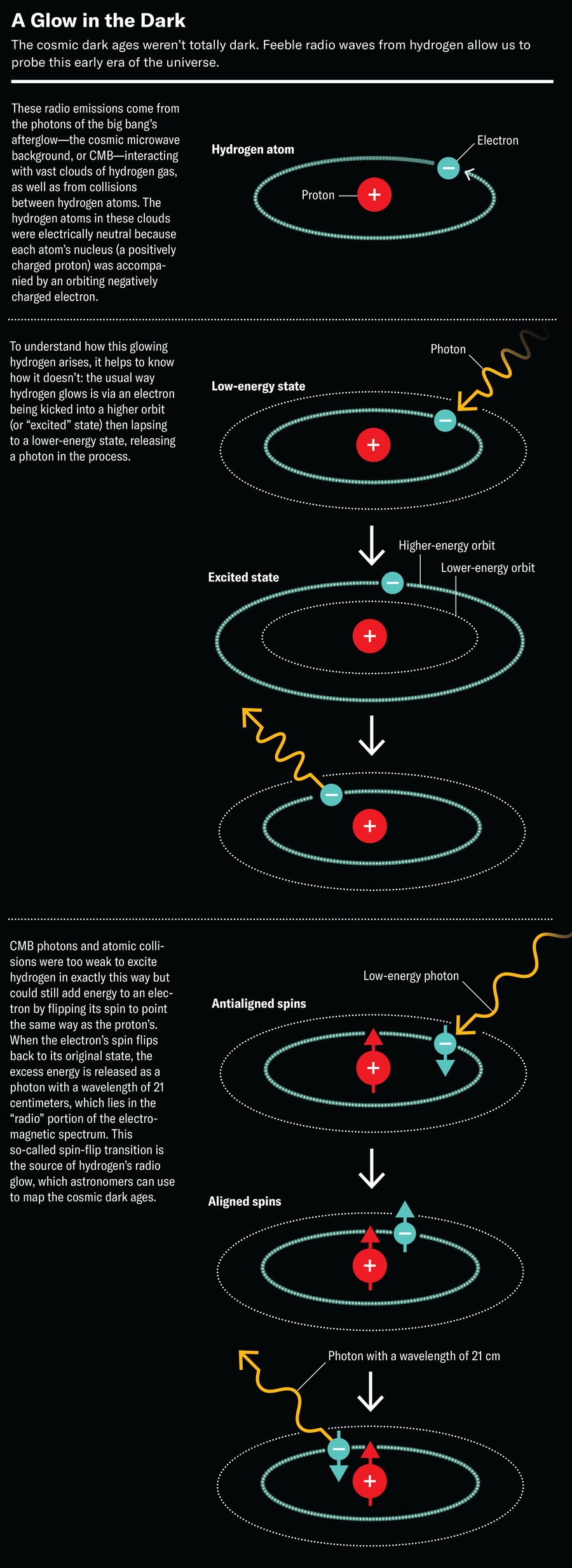
Furthermore, the environment in which the hydrogen atoms are embedded adds richness to the signal’s information content. The rate at which absorption or emission happens largely depends on the gas density, as well as the background ultraviolet and x-ray light produced by stars and black holes. These and various other factors each affect the strength of the 21-cm signal and imprint patterns upon it throughout the universe’s history. As a result, the 21-cm signal not only offers a view of the first luminous objects but also provides a way to trace the universe’s expansion and evolution across cosmic time.
The complex dependence of the 21-cm signal on these physical processes makes it an excellent astrophysical probe. For example, the ultraviolet radiation from the first stars interacts with hydrogen to imprint bubbles in the 21-cm signal with a radius of circa 100 million light-years. This interaction occurs because the starlight affects the rate of the spin-flip transition, coupling it to the motions of hydrogen atoms, which in turn are dictated by the hydrogen gas’s temperature. The first black holes also affect the signal as they produce x-rays from the glowing disks of matter that build up around them as they feed. These energetic photons heat up the gas around each source, imprinting large-scale fluctuations in gas temperature, which are mirrored in the 21-cm signal’s intensity.
Collectively, such effects form a rich tapestry of 21-cm radiation that offers a detailed picture of the early universe—but the signal eventually fades away, washed out by the ionizing radiation of galaxies. As galaxies grow bigger and more massive, the floods of ultraviolet photons they unleash boil away most of the remaining primordial, neutral hydrogen, breaking it back down into a plasma of charged particles. These charged particles are unable to exhibit the spin-flip transition, and so as the universe reionizes, the cosmological 21-cm signal is lost. Smaller and more localized 21-cm emissions can endure, however, emanating from islands of dense neutral gas cocooned within galaxies and shielded from the ionizing radiation.
Whispers of a Signal
But is this promising 21-cm signal really within our reach, and do we have the technology to detect it?
If we were to put a rarified cloud of hydrogen gas at cosmic density in a laboratory here on Earth, the 21-cm signal would not be observable because it takes roughly 10 million years for an individual hydrogen atom to spin-flip. Fortunately, the universe has existed for a much longer time. The universe’s enormously large number of hydrogen atoms further increases the imprint of this weak transition. It has already been demonstrated, for example, by the Canadian Hydrogen Intensity Mapping Experiment (CHIME) radio observatory and the MeerKAT array in South Africa, that the cosmological hydrogen signal can be detected from islands of neutral gas in the nearby universe.
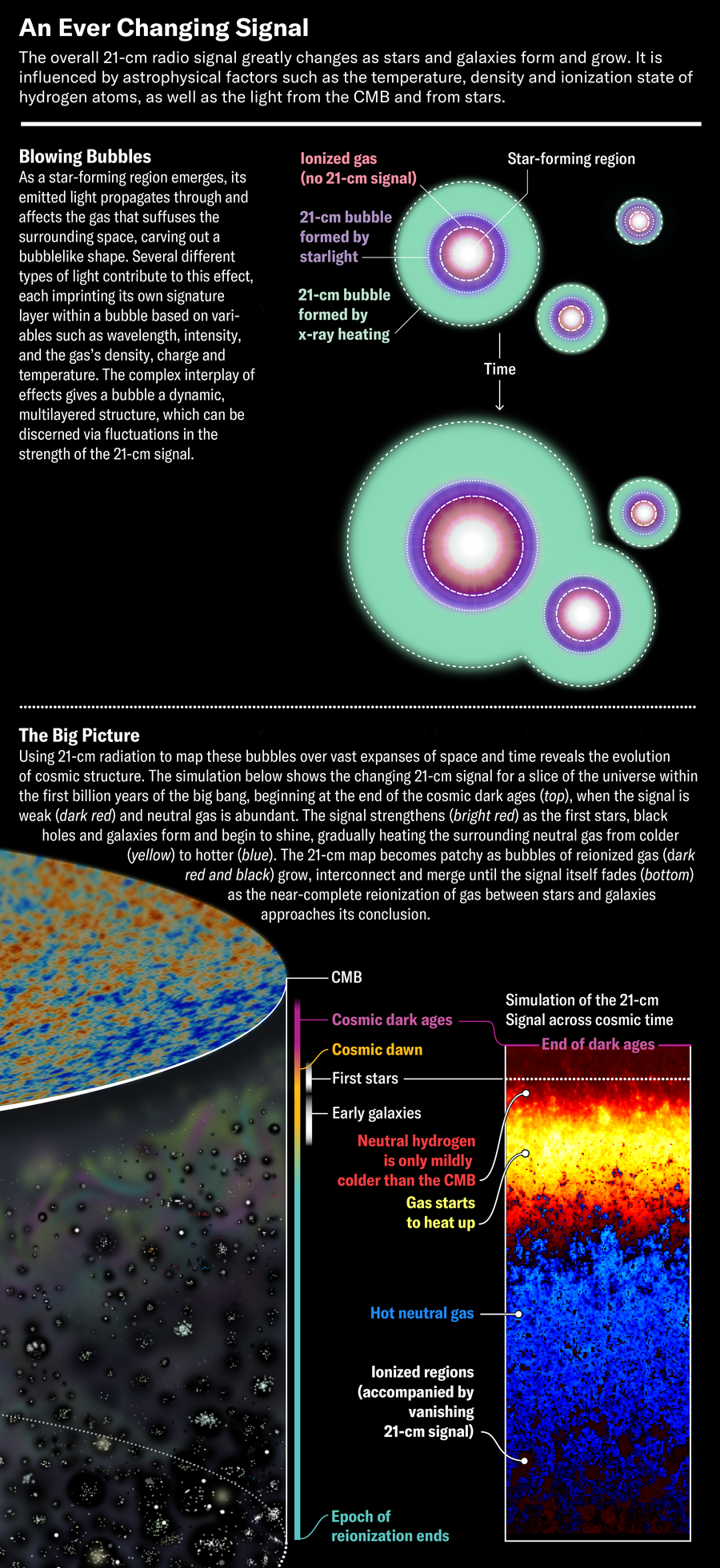
The wavelength of the 21-cm photons carrying the signatures of the first stars, black holes and early galaxies is stretched by the expansion of the universe, a phenomenon known as cosmic redshift. Observing this cosmically stretched signal of the very first stars thus requires detecting radio waves with wavelengths of several meters. Unfortunately, such wavelengths partially overlap with the popular FM radio band. In other words, your love of listening to loud music on the radio also helps drown out the ancient whispers of the universe’s first stars.
Even without human-made transmissions, observing the relatively weak 21-cm cosmological signal is very challenging because it is swamped by emissions several orders of magnitude stronger. These emissions come from electrons whirling within powerful magnetic fields snaking through and around the Milky Way and other galaxies. Measuring the signal at all is like hearing someone’s faint breathing in a room where a loud siren is blaring. But sophisticated data-analysis algorithms, some based on machine-learning methods, should be able to pierce this veil of overlying astrophysical noise to extract the faint signal from primordial hydrogen at early cosmic times.
Researchers have devised two major approaches for seeking this faint signal. The first uses a single compact antenna that can measure the total 21-cm signal from all across the sky. Such experiments are said to measure the “global” signal, and they probe milestone events of cosmic history such as the onset of star formation, heating and reionization. In 2018, to the excitement and surprise of the scientific community, a lone radio telescope in the Australian Outback called Experiment to Detect the Global Epoch of Reionization Signature (EDGES) delivered the first tentative result from this approach, an all-sky measurement of the 21-cm signal from 200 million years after the big bang.
The absorption signal reported by EDGES was surprisingly deep, suggesting that either the gas was significantly cooler than predicted—a potential sign of an unexpected interaction of ordinary matter with the colder dark matter—or that the background radiation was much stronger than the CMB. This latter possibility would require unusually bright radio sources at cosmic dawn—or perhaps some exotic particle physics mechanism producing excess photons with a 21-cm wavelength. A more recent follow-up by another global 21-cm experiment, a radio telescope called Shaped Antenna Measurement of the Background Radio Spectrum 3 (SARAS 3) deployed on a large lake in southern India, failed to validate the EDGES low-band result. But, as testament to the heroic difficulty of these measurements, uncertainty remains as to which result is correct. The EDGES and SARAS teams, among others, are continuing their efforts to constrain the sky-averaged 21-cm signal from the epoch of reionization and cosmic dawn, as are several other collaborations, such as the Radio Experiment for the Analysis of Cosmic Hydrogen (REACH) collaboration co-led by the University of Cambridge and Stellenbosch University in South Africa.
The second method uses large antenna arrays called interferometers to seek spatial fluctuations in the 21-cm signal rather than its mere all-sky presence and strength. Interferometers record differences in power across the sky, thus measuring the signal’s location-based variance at any given instant in cosmic history. This signal contains far more spatial information compared with the global one and can reveal otherwise-unobtainable details about the early universe such as the distribution of the first star-forming regions across the sky. Low-frequency interferometers—such as the Low-Frequency Array (LOFAR), the Hydrogen Epoch of Reionization Array (HERA), the Murchison Widefield Array (MWA) and the Large-Aperture Experiment to Detect the Dark Ages (LEDA)—have reported upper limits on the power spectrum of fluctuations in the 21-cm signal from cosmic dawn and reionization and are collecting more data. The 21-cm cosmology community awaits the Square Kilometer Array (SKA), which is now being constructed in Australia and South Africa. SKA is expected to go further than the existing telescopes creating images at a large range of redshifts, thus providing a tomographic three-dimensional scan of neutral hydrogen in the early universe.
In the grand quest to unveil the universe’s dark ages, the final frontier for measurements of the global and spatial cosmological 21-cm signals alike may well be the far side of Earth’s moon, where our natural satellite’s bulk blocks out earthly interference. Multiple proposals already exist for radio arrays on the lunar far side, and such projects could be pursued—or precluded—as part of the international race to send humans back to the moon later this century. But whether on Earth or off-world, we find ourselves on the cusp of yet another transformative breakthrough, watching the shadows of uncertainty fade in the final moments before we see, at last, the break of cosmic dawn.
Leave a Comment